Even though I am a strong advocate of public transportation for my daily commute to and from work, I am still heavily reliant on my car. Even though it´s still powered by a conventional engine, I am convinced that the next step will be an electric car. In this transition to electric mobility, it is fascinating to see how the analytical tools I have been working with for years can help researchers and developers to improve battery performance, lifetime, and security. Let me share a few aspects where I see gas chromatography mass spectrometry (GC-MS) has been key in battery development.
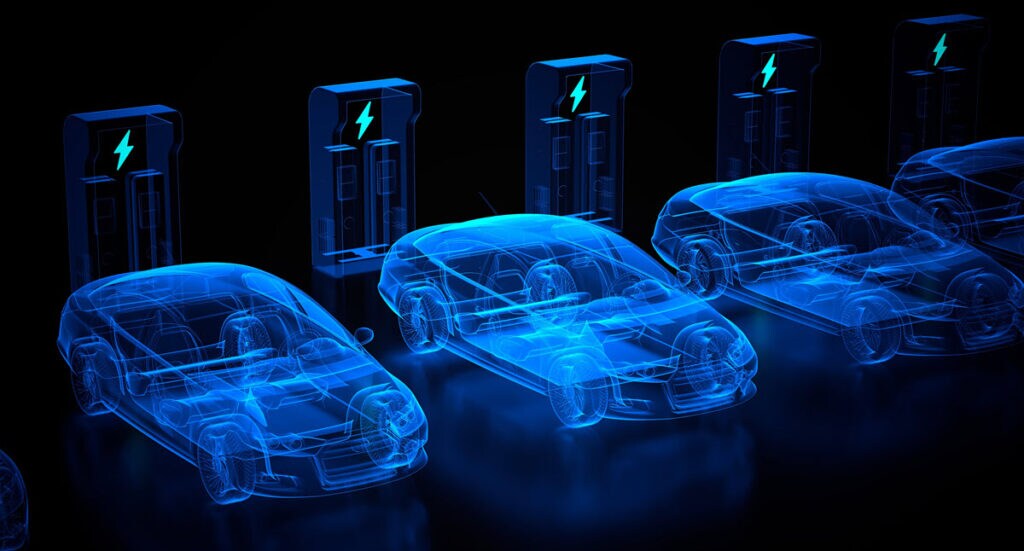
A typical lithium-ion battery consists of four main parts, namely the cathode, separator, anode, and electrolyte (Figure 1). While the elemental composition of cathode and anode materials can be analyzed using Inductively Coupled Plasma Optical Emission Spectroscopy (more on this in a previous blog post), today we will focus today on GC amendable analytes, such as those found in the composition of the electrolyte.

The most common electrolyte used in LiBs is lithium hexfluorophosphate (LiPF6) salt mixed with volatile organic carbonate solvents (i.e., dimethyl carbonate, ethyl methyl carbonate, diethyl carbonate).
Upon the first cycling of the LiB, reduction of the electrolyte at the anode surface produces a conductive film known as the solid electrolyte interface (SEI), where current (i.e., Li+) can pass through while simultaneously preventing further reduction of the electrolyte. However, the LiPF6 is thermodynamically unstable at elevated operating temperatures (> 60 °C), causing the organic carbonate solvents to be further reduced and limiting the battery’s current flow and charging capacity.
The analysis of the electrolyte composition is therefore important to diagnose the cause of a battery’s failure to perform to designed specifications. For analysis of the major components (present at percent volume concentrations), require dilution factors of at least 10,000. At the same time, samples need to be analyzed almost undiluted for analysis/screening of minor components and electrolyte degradation products. This means that an analysis needs to cover a huge concentration range, to ensure that compounds in trace level concentrations can still be detected, while avoiding detector saturation by the major components.
Another obstacle is the removal of the LiPF6 salt prior to analysis by GC to help protect the column and MS system. In addition, hydrofluoric acid (HF) can be produced when trace amounts of moisture are present, making removal of the PF6– essential prior to analysis to avoid formation of HF in the injection system1. Salt removal can be accomplished using dichloromethane (CH2Cl2), followed by centrifugation. Once this is accomplished, the electrolyte can be analyzed using different GC-MS systems.
Analysis using different GC-MS systems
Single quadrupole GC-MS, like the Thermo Scientific™ ISQ™ 7610 GC-MS system, can reliably analyze the electrolyte composition and provide information on major components, additives and degradation products.
Triple quadrupole, or GC-MS/MS systems, such as the Thermo Scientific™ TSQ™ 9610 GC-MS system, can provide higher sensitivity and selectivity. Targeted methods screening for specific components can screen for known degradation products in a larger scale experiment.
High resolution accurate mass (HRAM) systems, like the Thermo Scientific™ Orbitrap™ Exploris GC 240 GC mass spectrometer allow for non-targeted analysis, and can help with identification of degradation products and/or mechanistic elucidation of processes that lead to performance loss. The sample preparation can be fully automated using the Thermo Scientific™ TriPlus RSH Smart Autosampler. This advanced robotic sample handling system can be fitted with all required components, including a vortex mixer and a centrifuge. The system can process 54 samples completely unattended, with the analysis being conducted online shortly after the removal of the salt has been accomplished. The process is fully overlapping with the subsequent GC-MS analysis, meaning that the next sample is prepared while the current injection is being analyzed. Here´s a video that shows the process:
Another emerging application for GC-MS can be found in battery recycling. Batteries having reached the end of their lifespan are often shredded and further processed to obtain valuable materials needed for new battery construction. Due to the complexity of LiBs, efficient separation of the various components is critical to maximize recovery of essential metals. This is particularly important for organic binders, as their presence in LiB shred material can reduce essential metal extraction efficiency during the recycling process.2 This can be accomplished using pyrolysis, either coupled with single quadrupole GC-MS or HRAM. A common binder material is PVDF, which during the pyrolysis process decomposes to a variety of pyrolyzates, including fluoroalkenes with various chain lengths, subsequently undergoing in-source fragmentation to produce specific molecular markers, such as 1, 1, 3, 3-tetrafluroallylium (C3HF4+, m/z 113.0009).
However, in this mass range, ions formed by other compounds could appear as isobaric interferences, leading to false positive identifications. At a a mass accuracy setting of 500 mmu (typically achieved in single quadrupole GC-MS instrumentation), isobaric interference from the sample matrix is clearly visible (see Figure 2). Using higher mass resolution (60,000 at 200 m/z full width half maximum) and accuracy (5 ppm) settings obtained with the Orbitrap Exploris GC mass spectrometer, this interference is easily removed and simplifying identification with standard material. This highlights the selectivity advantage of high-resolution accurate mass instruments, dramatically simplifying the spectral identification of binder materials.
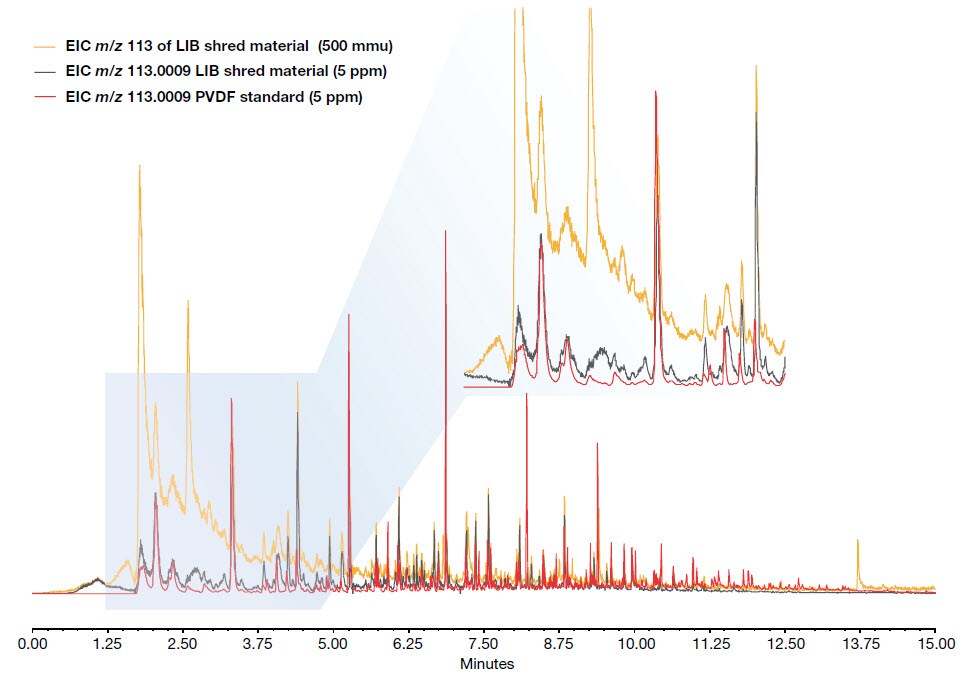
For more information on the application of gas chromatography mass spectrometry in the field of lithium batteries, please visit this website!
References
1: Kösters, K., Henschel, J., Lürenbaum, C., Diehl, M., Nowak, L., Winter, M., Nowak, S.: Fast sample preparation for organo(fluoro)phosphate quantification approaches in lithium-ion battery electrolytes by means of gas chromatographic techniques; Journal of Chromatography A 2020, 1624, 461258
2: Petranikova, M.; Naharro, P. M.; Vieceli, N.; Lombardo, G.; Burçak, E. Recovery of critical metals from EV batteries via thermal treatment and leaching with sulphuric acid at ambient temperature. Waste Management 2022, 140, 164–172.
On LinkedIn? Visit our LinkedIn page #BatteryTesting #GCMS